Native starch is a good texture stabilizer and regulator of food systems, but factors such as its low shear resistance, thermal resistance, and high tendency to retrogradation restrict its use in some food applications. Starch is commonly modified both chemically and physically to generate starches with special functional properties. However, most industries (especially the food and pharmaceutical industries) prefer starches that have been physically altered, to ensure their relative safety. Slowly Digestible Starch (SDS) can also be prepared with enzymatic methods or several of these methods.
- Introduction to Slowly Digestible Starch (SDS)
- Digestibility of Slowly Digestible Starch (SDS)
- Physicochemical Properties of Slowly Digestible Starch (SDS)
- Functions of Slowly Digestible Starch (SDS)
- Applications of Slowly Digestible Starch (SDS)
Physical Modification Methods
The advantages of using physical methods to prepare Slowly Digestible Starch (SDS) are that these methods are considered more natural and are very safe. The physical modification methods used to produce SDS include hydrothermal, malleablization, autoclaving, microwaving, and polymer entrapment methods (Table 1). The SDS content of pea starch is the highest of the native starches. Heat–moisture treatment (HMT) markedly improves the SDS content of waxy potato, potato, waxy corn, rice, yam, and banana starches, whereas it has little obvious effect on pea starch.
Modified method | Native starch | Preparation condition | Native starch* | Modified starch* |
HMT | Waxy potato | Water 25.7%, 120°C, 5.3h | 8.1 | 41.8 |
HMT | Pea | Water 30%, 120°C, 24h | 40.3 | 45.3 |
HMT | Potato | Water 30%, 30°C, 12h | 5.4 | 37.5 |
HMT | Waxy corn | Water 35%, 120°C, 10h | 0.8 | 9.3 |
HMT | Germinated brown rice | Water 30%, 100°C, 1h | 39.1 | 46.3 |
HMT | Rice | Water 16%, 121°C, 1h | 38.2 | 43.3 |
Malleableize | Pea | Water 70%, 50°C, 24h | 40.3 | 43.2 |
Autoclaving | Yam | Water 83%, 121°C, 1h | 15.0 | 34.1 |
Parboiling | Corn | Water 83%, 60°C water bath 2h | 22.2 | 35.5 |
Microwave | Banana | Water 83%, 20 min | 9.9 | 18.3 |
β-Cyclodextrin | Rice | Water 80%, 25 °C, β-CD 3% | 18.1 | 52.1 |
A higher percentage of Slowly Digestible Starch is mainly attributed to the facts that intact starch granules are retained during physical modification treatments and that intact granules are less susceptible to amylolytic enzymes. Some treatments cause the formation of amylose–lipid complexes in the starch granules, which can lower the susceptibility of SDS products to enzymatic degradation. In other words, any physical modification of the starch structure that affects enzyme binding and therefore the rate of its digestion can be used to modulate starch digestibility and form SDS.
On the other hand, a higher percentage of Slowly Digestible Starch can involve a higher ratio of imperfect crystallites to perfect crystallites. Most SDSs consist of amorphous regions and weak crystallites, with a high proportion of dextrin, with a degree of polymerization (DP) ≥ 25. This structural information can be used to develop low-digestibility food products.
Figure 1 shows the surface features and cross-sectional views of HMT
waxy potato starch granules. Scanning electron micrographs of the native starch
granules show round or oval shapes, with no evidence of fissures or cracks
(Fig. 1a1), and the cross sections show no hollow internal structures (Fig. 1a2).
However, the surfaces of all the HMT samples show signs of cracking and dents
(Fig. 1b1–f1), and the cross-section of each starch granule shows a large hollow in the central region, the size of which is probably proportional to the moisture level. These results may be attributable to the partial swelling and disruption of the starch granules by the abundant water molecules. The hollow structures at the centers of the HMT starch granules may have resulted from the rearrangement of the molecular structure and the disintegration of the central tissue during HMT. At a high moisture level (30%), the hollow region was large and readily visible.
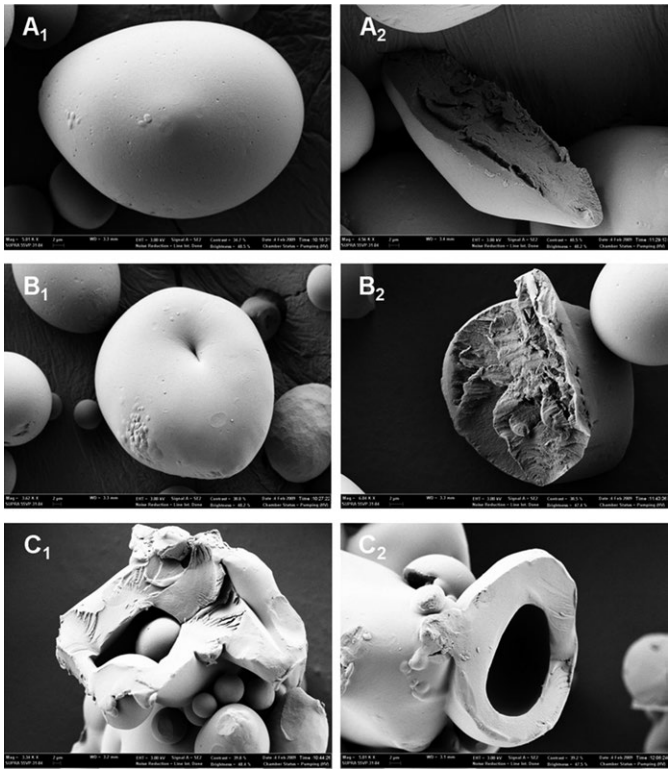
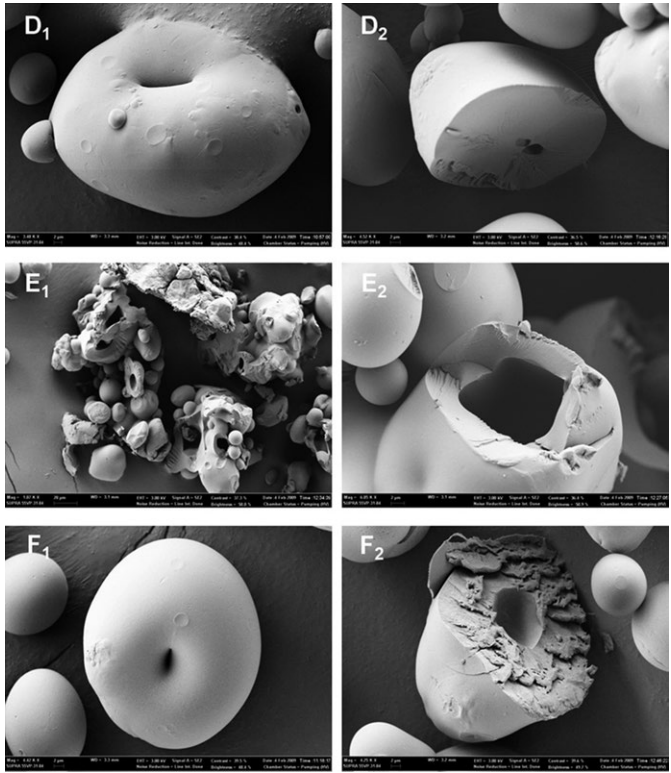
Both samples B and D were treated with 20% moisture. No hollow region was observed in the cross-section of starch sample B (110 °C, 5 h), but a small hollow region was observed in sample D (130 °C, 9 h). Therefore, this difference is attributable to the higher treatment temperature and longer treatment time. Samples C (130 °C, 1 h) and E (150 °C, 5 h) were both treated with 30% moisture and showed the largest hollow regions. Overall, the size of the hollow region increased as the moisture level, temperature, and time of treatment increased. Among these three factors, the moisture level had the most significant effect on the size of the hollow region.
The development of new crystallites in the amorphous region through the interactions between amylose chains or the formation of crystalline amylose–lipid complexes may contribute to the reduction in enzyme sensitivity of HMT starches. Recent studies have demonstrated that the hydrophobic section of some lipids is preferentially introduced into the central axis of the amylose helix to form an amylose–lipid complex during interactions between amylose and lipids. The amylose chain of starch has a natural twist, producing a helical conformation with six anhydroglucose units per turn. Amylose has a helical conformation and can form inclusion complexes with small hydrophobic molecules. Complexes between fatty acids, such as lauric acid, and amylose can form rapidly under physiological conditions and contribute to the formation of both Slowly Digestible Starch (SDS) and Resistant Starch (RS). The formation of such complexes with lipids can cause significant changes in the behavior of starch, reducing its solubility, increasing its gelatinization temperature, delaying its retrogradation, and increasing its resistance to digestive enzymes.
The complex formed has an unstable V-type crystalline structure and inhibits the formation of B-type recrystallized starch. The stability of the V-type complex to amylolytic and lipolytic enzymes has been estimated, and its melting temperature is above 100 °C. This resistance to high temperatures protects Slowly Digestible Starch (SDS) from dissociation during food processing. However, the lipid content added during the formation of amylose–lipid complexes often exceeds 10% and contributes a lot of additional energy.
Zhan et al. demonstrated that β-cyclodextrin (β-CD) interacts with paddy starch to increase the yield of SDS. Under the optimum conditions for modification (β-CD, 3%; water, 80%; and equilibrium temperature, 25 °C), the maximum SDS yield was 52.1%. The basic rule for conferring slow digestibility is the formation of starch– β-CD non-inclusion complexes with a partial V-type structure and weak resistance to enzymes. Starch–β-CD non-inclusion complexes were found more suitable for improving the SDS yield than starch–lipid complexes. In vivo data have suggested that potato amylose–lipid complexes (weight ratio, amylose/lipid = 5:1, 60 °C) are hydrolyzed and absorbed within 120 min of ingestion to the same extent, but somewhat more slowly, than uncomplexed starch, with a 12% lower digestion rate.
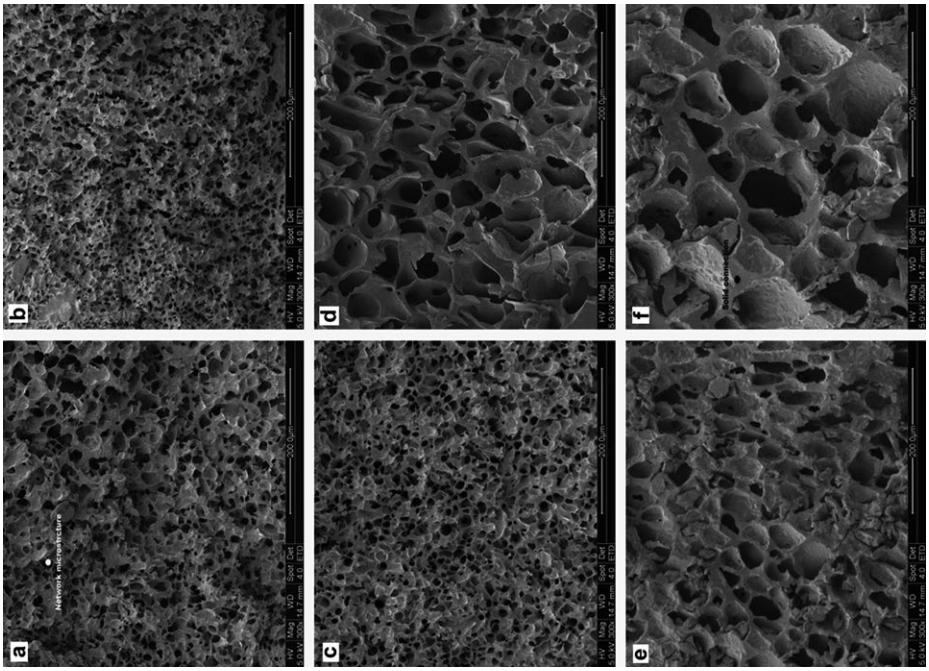
Figure 2 presents SEM images of a rice Slowly Digestible Starch (SDS) product prepared with single-retrogradation or dual-retrogradation treatments over different time intervals. In that study, rice starch (5.0 g) was dispersed with two volumes of distilled water and heated in a boiling water bath for 30 min. The resultant gel was hermetically sealed and stored at 4 °C for 24, 36, or 48 h to analyze its retrogradation. The retrograded samples were pregelatinized in a boiling water bath for 20 min and subjected to a dual-retrogradation treatment for periods of 24, 36, and 48 h. Each of the resulting gels was dried at 40 °C for 8 h. The SEM images revealed that the SDS products that underwent single retrogradation had network microstructures with smaller cavities than those observed in SDS after dual retrogradation. This morphological conformation was produced by starch gelatinization but was not affected by the retrogradation time (Fig. 2 a, e, c). However, larger holes and more solid connecting parts were observed in the SDS products subjected to dual retrogradation (Fig. 2). The larger cavities reduced the contact area between the starch and amylase molecules. Furthermore, the solid connecting parts formed during the dual-retrogradation treatment prevented the starch molecules from leaching out and produced moderate retrogradation, resulting in a higher SDS yield. Compared with single retrogradation, dual retrogradation generated larger cavities and more solid connection parts in the SDS products. This internal microstructure increased the degree of slow digestibility. These findings suggest that dual retrogradation can be used to increase the yield of SDS in starchy products.
Chemical Modification Methods
Starches are modified by chemical methods to improve their functionality and increase their commercial value. Several key structural properties of starches can be modified to functionalize the copolymer to meet specific requirements and to confer a variety of physicochemical benefits.
Many modified starches made for food use contain only small amounts of substituent groups and are used as safe food ingredients. Legislative approval for the use of novel starch derivatives in processed food formulations is still under debate, but several tailor-made starch derivatives with multiple modifications are being prepared and characterized. Some chemically modified starches are increasingly used as fat replacements or fat substitutes in different food systems. These starches are either partially or totally undigested and therefore contribute few calories to the food.
The chemical modification of starch is generally achieved through its derivatization, including the etherification, cross-linking, oxidation, substitution, and grafting of the starch molecule. The chemical modification involves the introduction of functional groups into the starch molecule, which markedly alters its functional properties. These modifications of native granular starches profoundly alter their gelatinization, pasting, and retrogradation behaviors.
Reagents | Native starch | Preparation condition | Native starch* | Modified starch* |
Citric acid | Paddy rice | 2.62 mmol acid/20g starch, 128.4°C, 13.8h | 9.8 | 23.0 |
Citric acid | Mung bean | Citric acid 30%, starch 20%, pH 2.0, 120°C, 1h | 15.1 | 23.3 |
Hydrochloric | Mung bean | Hydrochloric 1.0 mol/L, starch 20%, pH 5.0, 120°C, 1h | 15.1 | 23.3 |
Vitriol | Mung bean | Vitriol 0.3 mol/L, starch 20%, pH 2.0, 120°C, 1h | 15.1 | 23.3 |
OSA | Waxy corn | OSA 3%, starch 40%, pH 8.4, 35°C, 2.3h | 15.0 | 25.0 |
OSA | Waxy corn | OSA 3%, starch 57%, pH 8.5, 20°C, 6h | 15.3 | 28.1 |
Some chemical methods used to prepare Slowly Digestible Starch (SDS) are shown in Table 2. After treatment with acid or esterification, the SDS content of the modified starch is significantly greater than that of the native starch.
Chemical reagents provide nonionic, cationic, hydrophobic, or covalently reactive substituent groups. These modifications generally alter the gelatinization and pasting properties of starch. Citric acid, a polyfunctional carboxylic acid, can be used to esterify the hydroxyl groups on starch, resulting in the formation of cross-links and improving the starch properties or SDS content.
A study of paddy rice starch treated with citric acid (2.62 mmol acid/20 g starch, 128.4 °C for 13.8 h) showed an increase in the apparent amylose content from 21.1% to 30.3%, indicating that it contained more linear chains, derived from the amylopectin side chains and acid-hydrolyzed amylose. The reduced molecular weight caused by the acid treatment allowed greater freedom of polymer motion and enhanced its ability to form more stable structures that better resisted enzymatic hydrolysis.
Esterification with octenyl succinic anhydride (OSA) is one of the modifications that most effectively increase Slowly Digestible Starch (SDS). OSA-modified starch showed an extremely low glycemic response during human trials, consistent with the extended glucose release profile of SDS. The SDS (42.8%) produced by subjecting OSA-treated waxy corn starch to HMT (10% moisture, 120 °C for 4 h) was higher than that produced by treating OSA-modified starch with the Englyst test method (28.3%). The modified starch products with attached OSA molecules may act as noncompetitive inhibitors of digestive enzymes, reducing enzyme activity and thereby slowing digestion. As these studies show, chemical modification can be used to prepare SDS, but clinical and toxicological trials are required to evaluate the safety and nutritional efficacy of consuming this SDS.
Enzymatic Modification Methods
The enzymatic processing of starch is a commonly used and effective modification technique. Enzymatic treatments of starch with pullulanase, isoamylase, α-amylase, β-amylase, or transglucosidase can change the chain lengths of starch, thus achieving the appropriate digestibility and glycemic response.
Enzymes | Native starch | Preparation condition | Native starch* | Modified starch* |
Pullulanase | Waxy rice | Starch (10% w/v) boiled with continuous stirring for 30 min, adjust the temperature to 58°C, pullulanase 60 ASPU/g, 12 h | 45.5 | 57.8 |
Amylosucrase | Waxy rice | Starch (2%, w/w) and sucrose (100 mM) in 100 mM in sodium citrate buffer (pH 6.0), boil suspension for 10 min. Adjust temperature to 30°C, add amylosucrase (40,000 U), 40h | 4.9 | 29.1 |
Amylosucrase | Waxy corn | Ditto | 5.2 | 30.2 |
Amylosucrase | Waxy potato | Ditto | 4.4 | 24.2 |
β-Amylase | Normal corn | Starch (8% w/v) shake for 20 min in 95°C, adjust temperature 55°C, pH 5.2, adding 0.032% β-amylase, shake for 12 h | 15.2 | 22.4 |
β-Amylase+transglucosidase | Normal corn | Starch (8% w/v) shake for 20 min in 95°C, adjust temperature 57°C, pH 5.2, adding mixed enzyme (composited ratio, 0.032%:8 TGU), shake for 8 h | 15.2 | 33.5 |
Maltogenic amylase | Normal corn | Starch (8% w/v) shake for 20 min in 95°C, adjust temperature 60°C, pH 4.8, adding 40 mg/kg maltogenic amylase shake 16h | 15.2 | 38.1 |
After treatment with different kinds of enzymes, the structures and physicochemical properties of starches change. Some enzymatic methods used to prepare Slowly Digestible Starch (SDS) are shown in Table 3. After treatment with enzymes, the SDS contents of most starches clearly increase. After treatment with pullulanase, the SDS content of native waxy rice starch reached a high level but decreased to 24.9% after cooking.
Xiong studied the Slowly Digestible Starch (SDS) content of normal corn starch after β-amylase, transglucosidase, or maltogenic amylase treatment. The product obtained after processing with β-amylase showed an increased SDS content, with an average chain length of DP 16.16 and a branch density of 6.19%. After treatment with a combination of enzymes (β-amylase and transglucosidase), the product had a higher SDS content, with an average chain length of DP 14.36 and a branch density of 6.96%. After treatment with maltogenic amylase, the product had the highest SDS content, with an average chain length of DP 10.95 and a branch density of 9.13%. These results suggest that enzymatic treatments can increase SDS contents and that treatment with maltogenic amylase is most effective.
The molecular weight of most starches decreases rapidly during enzymatic treatment. According to BeMiller and Whistler, a native starch formed two fractions with different molecular weight: amylopectin, which is a larger molecule (107 –108 ), and amylose, which is a smaller molecule (104 –106 ).
The addition of enzymes reduced the contents and molecule weights of both amylose and amylopectin. According to Christophersen, maltogenic α-amylase quickly reduced the peak DP of amylose, with the formation of only minor glucose, maltose, and other low-molecular-weight oligosaccharides. They reported that only 3% of other low-molecular-weight oligosaccharides were produced, but the DP was dramatically reduced from DP 350 to DP 123. Bijttebier et al. reported that the maltogenic α-amylase from Bacillus stearothermophilus preferentially hydrolyzed the exterior chains of amylopectin during the early stages of hydrolysis but also hydrolyzed the inner chains, with high multiple attack action, during the later stages. These data suggest that the endomechanism of maltogenic α-amylase is consistent with the reduced molecular weight of the starch molecule. It is generally known that amylopectin consists of multiple clusters connected by long linear chains. Based on the enzymatic properties of the branching enzyme, the α-1,6- linked segments between the amylopectin clusters are hydrolyzed to release the cluster units. Similar findings have been reported by the others. The action of enzymes on the linear chain of amylose can also form branched linkages and a cyclic amylose, and these reactions may change the physical structure of the substrate, slowing its digestion time.
According to the Hizukuri cluster model, amylopectin molecules have A, B (B1– B4), and C chains, and the fractions DP < 13 and DP 13–30 together constitute the short chains, corresponding to the A + B1 chains. The other longer-chain fractions correspond to the B2–B4 chains. It has been reported that maltogenic α-amylase has a marked effect on the side-chain distribution of starch and especially reduces the number of short B chains. In that study, maltogenic α-amylase reduced the levels of the outer chains (primarily A and B1) by 50% compared with the control sample, with little effect on the internal chain length. Based on these observations, it can be concluded that the addition of maltogenic α-amylase reduced the molecular weight of amylopectin, and significantly affected the side-chain distribution of the residual amylopectin, increasing the relative numbers of short chains. The reduced proportion of short chains could lead to the formation of more perfect crystallites, increasing the starch’s resistance to starch-digesting enzymes.
In the study by Miao et al., gelatinized cornstarch (10% w/v, pH 5.0) was treated with maltogenic α-amylase (5 U/g dry weight of starch, 55 °C). The product had a higher proportion of short chains than the native cornstarch (44.2% and 23.7%, respectively), which maximized the Slowly Digestible Starch (SDS) content (19.6%). A starch debranching analysis (mutant cornstarch debranched with isoamylase) revealed a parabolic relationship between the SDS content and the weight ratio of amylopectin short chains (DP < 13) to long chains (DP > 13). Amylopectin with higher amounts of either short chains or long chains can contain relatively large amounts of SDS.
Composite Modification Methods
Compared with the modification methods discussed above, the composite modification methods have several advantages in the preparation of Slowly Digestible Starch (SDS), including greater efficiency and cost-effectiveness. Some composite modification methods used to prepare SDS are shown in Table 4, including different combinations of physical, chemical, and enzymatic methods. The combination of different kinds of modification methods has several advantages, including a great increase in the SDS content compared with the native starch.
Modified method | Native starch | Preparation condition | Native starch* | Modified starch* |
Pullulanase-retrogradation | Waxy corn | Native starch samples hydrolyzing with pullulanase (20 U/g) for 6h and then cooled at 4°C for 2 days | – | 45.1 |
Pullulanase-lipid | High amylose corn | Native starch slurry (10% w/v, pH 4.4) cooked in boiling water bath with stirring for 30 min. Adjusted temperature to 60°C, add pullulanase 40 ASPU/g of starch for 2 h; mix with lauric acid (10% w/w, db), boiling water bath for 30 min | 6.6 | 11.2 |
Emulsifier-alcohol | High amylose corn | Native starch 5 g, glycerin monostearate 0.5 g, 40% ethyl alcohol 35 mL, KOH (3 M) 25 mL cooked in 35 °C water bath for 15 min | – | 67.4 |
Cross-link-esterification | Waxy rice | Native starch slurry (35% w/v, pH 9) with STMP 6.5% cooked in 35 °C water bath for 4.5 h; adjusted to pH 8, add OSA 5.3%, cooked for 15 h | 13.8 | 33.8 |
Ultrasonic wave-pullulanase | Corn | Native starch slurry (10%) cooked in 70 °C water bath for 20 min; adjusted to 40 °C, add 14% pullulanase, ultrasonic wave (300 W) for 40 min | 25.3 | 43.1 |
Microwave-HMT | Corn | Water 60%, 50 °C, microwave 300 W for 25 min | 25.3 | 38.3 |
Dual modification methods combining debranching modification and other types of modification can be used to prepare Slowly Digestible Starch (SDS) with various properties and functionalities. Debranched-acetylated starch and debranched-octenyl succinylated (OSA) starch have shown great potential utility in starch–lipid complexes, low-fat salad dressings, and emulsion stabilizers. Recently, there has been increasing interest in the preparation of micro- and nanocomposites. These new composites, with slow digestibility and barrier properties, can be used to design economic and biocompatible delivery systems for bioactive agents in foods, beverages, and pharmaceutical agents.
In general, the physical modification methods for Slowly Digestible Starch (SDS) have several disadvantages, including lower yield. Moreover, the equipment required to produce SDS products is always expensive and cannot meet the requirements for continuous production. However, it is the safest way to generate SDS because no hazardous substances are incorporated into the products during processing. Chemical production methods for SDS are widely used in industry, but the use of chemical reagents in the production process is not environmentally friendly, and their application to food products is restricted. Enzymatic methods are suitable for producing many SDS products, but the cost is so high that they cannot meet the demands of industrial production. Together with the several other advantages of composite methods, the SDS produced with composite methods is more stable to heat than that produced by other methods.