At the turn of the last century the capability of high hydrostatic pressure to preserve milk in combination with moderate temperatures (Hite, 1899) and to denature egg albumin (Bridgman, 1914) has been discovered. Since that time, a lot of research has been carried out in the field of high hydrostatic pressure processing and many useful and pressure specific applications have been found. One of those utilisations of high pressure is the pressureassisted heating, especially of immiscible foods, where the temperature is increased almost homogeneously throughout the product due to the heat of compression. By this, one of the major drawbacks of conventional heating, where the temperature of the boundary layer of the product exceeds critical temperatures in order to reach the sufficient temperature for microbial inactivation in the centre of the product, can be overcome (Heinz & Knorr, 2002). High hydrostatic pressure is also a potential tool for the improvement as well as the generation of textural properties of food products.
Fundamentals
High hydrostatic pressure treatment is carried out batchwise or semi-continuously. The packed product is inserted into a cylindrical, low alloy steel vessel and pressure is built-up directly or indirectly. For direct, piston-type compression, the pressure medium inside the vessel is pressurised by a piston driven by a low pressure pump at the large diameter end of the piston. The pressure is transferred into the vessel by the small diameter high pressure vessel end of the piston where high pressure is generated by multiplying the low pressure by the ratio of the two piston diameters. For indirect pressurisation, a high pressure intensifier is used to pump pressure medium from a reservoir into the closed high pressure vessel. Thereby the same hydraulic principle of pressure generation is utilised by the intensifier as by the piston for the direct pressurisation (Deplace & Mertens, 1992).
Requirements for the packaging materials for high hydrostatic pressure treatment include flexibility, pressure tightness, heat sealability, by pressure unmodifiable mechanical and gas barrier, and non-toxic, aromatic-proof and sanitary properties (Masuda et al., 1992; Ochiai & Nakagawa, 1992).
Due to a resulting volume decrease upon pressurisation, any phenomena such as chemical reactions, phase transition or molecular configuration changes that are accompanied by a volume reduction are enhanced by high hydrostatic pressure according to Le Chateliers Principle. Consequently, high pressure affects non-covalent bonds (hydrogen, ionic and hydrophobic bonds) and some specific covalent bonds (Tewari et al., 1999).
The compression of the pressure medium and the food product is accompanied by heating, an increase in temperature of about 2-3°C/ 100 MPa occurs at adiabatic conditions. If it is aimed for isothermal conditions, the pressure vessel can be temperature controlled in order to dissipate heat upon compression and supply heat upon decompression. Otherwise a temperature field is formed because of slow heat conduction in comparison to a fast impulse transport of pressure acting uniformly throughout the vessel (Pfister et al., 2001). It is thus useful to integrate an indicator into the vessel that not only gives information about the pressure applied but also about the temperature conditions during the process.
Pressure time temperature integrator
With the growing demand in nutritionally valuable, fresh-like foods, conventional heating is no longer regarded sufficient to fulfil consumers’ demands because heating of potentially sensitive foods might lead to undesired changes in their nutritional, functional and organoleptic quality. Consequently, non-thermal decontamination methods for pasteurisation and sterilisation, which minimise the destructive influence of heat, are developed (Knorr & Heinz, 2001). Altogether, a microbiologically safe pressure treatment is possible (Smelt et al., 2002), for example Yen and Lin (1996) observed that guava puree treated at 600 MPa at 25°C for 15 min retained good quality after storage at 4°C for 40 days and Ogawa et al. (1992) found that citrus juice pressurised at 400 MPa at 40°C for 10 min was not spoiled during 2 to 3 months storage. However, control indicators depending on pressure, temperature and treatment time must be integrated into the process to evaluate the high pressure process and thus ensure microbiological safety and stability of the product and obtain regulatory approval.
In thermal processing the impact of the heat treatment is quantified by the application of so-called time temperature integrators (TTIs). These are heat sensitive components extrinsic or intrinsic to the food product that enable measuring directly and quantitatively the impact of the process without knowledge of the actual thermal history (Claeys et al., 2003). Such concepts are also required for pressure processes.
Claeys et al. (2003) investigated whether intrinsic components present or formed in milk enabled a direct and quantitative measurement of the impact of the pressure temperature process applied acting as pressure time temperature integrator (PTTI). The authors concluded that the data available regarding high pressure processing of milk was insufficient. Furthermore, Minerich and Labuza (2003) developed a pressure indicator for high pressure processing comprised of a compressed powdered copper tablet decreasing in density with increasing pressure and increasing treatment time. No significant impact of temperature on the copper density was found which limits the application of the copper tablets as PTTIs.
Pressurization of starch suspensions
Accessorily to the thermal gelatinisation, the crystalline order of starches can also be destroyed by mechanical means such as plastic deformation due to milling e.g. in a swing mill (Meuser et al., 1978) and high hydrostatic pressure treatment (French, 1984). A high pressure treatment up to 150 MPa increases the gelatinisation temperature (Thevelein et al., 1981), above 150 MPa the gelatinisation temperature is decreased (Muhr et al., 1982). Muhr and Blanshard (1982) found a gelatinisation of wheat starch in excess water at ambient temperature and 450 MPa. The pressure range in which gelatinisation occurs is typical for each starch (Stute et al., 1996) and partly depends on their crystalline structure e.g. B-type starches are more resistant to pressure than A- and C-type starches (Muhr & Blanshard, 1982; Ezaki & Hayashi, 1992; Stute et al., 1996; Rubens et al., 1999).
Snauwaert and Heremans (1999) and Rubens et al. (1999) observed pressure-induced starch gelatinisation in situ in a diamond anvil cell, Douzals et al. (1996) in a high pressure microscope. The authors discovered swelling of starch granules during pressurisation. Douzals et al. (1996) also observed further swelling of the granules i.e. an increase in granule volume after pressure release. The authors also detected a decrease in volume of the starch suspension during pressurisation, which partly remained after pressure release, and assumed that starch molecules linked with water occupy less volume than suspended in pure water and therefore the granule hydration would be preferential under pressure according to Le Chateliers Principle. Douzals et al. (1996) also viewed pressurisation of iodine stained starch granules and observed a decolouration of the swelling kernels which was regarded as an indication of starch melting.
Rubens et al. (1999) proposed a two step-mechanism for pressure-induced gelatinisation similar to the thermal gelatinisation process (Svensson & Eliasson, 1995). In the first step the amorphous regions were hydrated causing a swelling of the granules and a distortion of crystalline regions and in the second step of pressure-induced gelatinisation, the crystalline regions became more accessible to water. Thus under pressure a hydration of starch occurred before changes in crystallinity proceeded during gelatinisation.
As depicted in figure 2.9, the pressure-induced gelatinisation and the thermal gelatinisation differ in other respects e.g. evident by different rheological properties and microstructure of the starches (Stolt et al., 2001). Typical for most pressure-gelatinised starches e.g. as for wheat starch is the limited swelling of the melted granule (up to twice in diameter) and the maintaining of the granular character (Fig. 2.9). However, tapioca starch exhibits an exceptional swelling behaviour expanding six fold in diameter at 600 MPa. (Stute et al., 1996). Furthermore according to Douzals et al. (1998) there is only little and after Stute et al. (1996) and Stolt et al. (2001) sometimes even no amylose release. Pressurised starch suspensions are more condensed, with a different water binding capacity (Douzals et al., 1998). X-ray diffraction patterns of untreated and pressurised A-type starches in the presence of water show a transformation from the A- to the B-type X-ray diffraction pattern (Hibi et al., 1993; Katopo et al., 2002).
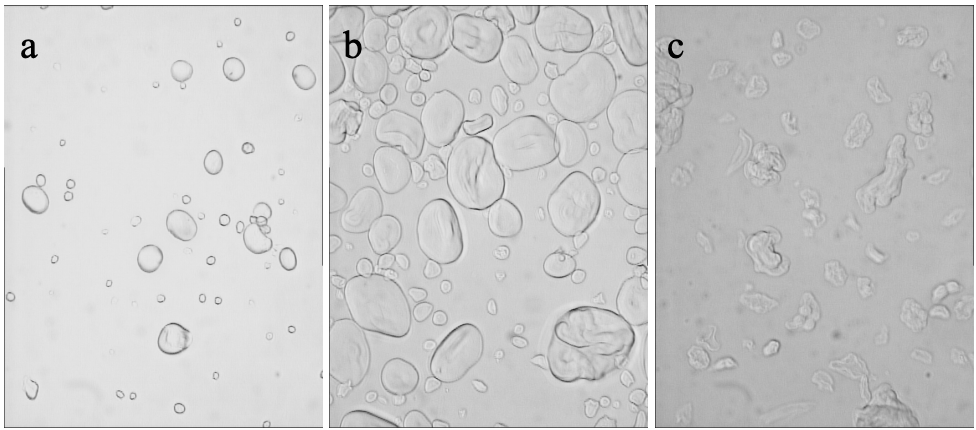
Moreover water plays an important role in the high pressure-induced gelatinization of starches. Suspended in alcohol even at very high pressures (up to 3 GPa) starch granules do not swell (Snauwaert & Heremans, 1999). Katopo et al. (2002) suggested that ethanol had a space filling effect stabilizing the crystallinity of starches. And according to Stute et al. (1996), a high moisture content is required for ultra-high pressure gelatinization. Other process parameters besides water content and type of starch influencing the gelatinization procedure are pressure, temperature and treatment time.
High hydrostatic pressure also influences the texture of starch suspensions or gels. The storage moduli of 10 % potato starch suspensions and 25 % barley starch suspensions increase with increasing treatment time at constant pressures until a constant G´ value is reached. And the higher the applied constant pressure, the faster the storage modulus increases with increasing treatment time (Stolt et al., 2001; Michel & Autio, 2003). For waxy maize starch suspensions, the storage modulus first increases and then decreases at pressures above 500 MPa with increasing pressurisation time (Stolt et al., 1999). The authors therefore assumed that excessive pressurisation weakens the structure of waxy maize starch gels. Stolt et al. (2001), investigating the effect of high pressure on barley starch suspensions with increasing treatment time, determined that consistency index, melting enthalpies and DSC peak temperatures increased with increasing pressure and pressurisation time. Consequently, a relationship between physical starch properties and pressure and time has been observed.
Hayashi and Hayashida (1989) reported an increased amylase digestibility of pressuretreated wheat starch at elevated temperatures relative to alkali treated starch. However, they also discovered the formation of a new, amylase resistant starch structure during long-term pressurisation (17 h). The glucoamylase digestibility rate of pressurised starches was also enhanced at elevated temperatures analysed immediately after pressure treatment and compared to raw starches but did not differ notably relative to thermally treated starches (Takahashi et al., 1994). However, the overall glucose yield by amyloglucosidase hydrolysis of gelatinised wheat starches was considerably improved by pressure-induced gelatinisation compared with thermal gelatinisation (Selmi et al., 2000). Ezaki and Hayashi (1992) observed a slower retrogradation of pressurised starches in comparison to thermally gelatinised starches determined by enzyme digestibility and iodo-starch reaction. These results were in accordance with findings made by Douzals et al. (1998). They discovered a quantitatively lower retrogradation of pressure-induced wheat starch gels showing to be less sensitive to ageing and less sensitive to storage conditions known to be favourable for starch retrogradation (4°C). This could open up novel applications of pressure treated starches in bread to reduce bread staling. On the other hand, Stolt et al (2001) observed comparable retrogradation behaviour of heat-induced and pressure-induced starch gels. DSC-investigations of pressurised wheat starch revealed the development of a retrogradation peak showing a faint Bpattern after a pressurisation step leading to the assumption of a rapid retrogradation during or instantaneously after the high pressure treatment (Stute et al., 1996). Katopo et al. (2002) presumed that a pressure-induced rearrangement of double helices had occurred in the A-type starch represented by this additional peak. It was therefore interesting whether the starch fraction causing this additional DSC peak observed immediately after pressurisation by Stute et al. (1996) and Katopo et al. (2002) contributed to the resistant starch (RS) content and thus could prove high pressure to be a feasible alternative for the RS production.